During last century, some high-profile designs suffered cataclysmic failures as a result of naturally occurring frequencies not being calculated, says Laurence Marks. While we have the tools to calculate them today, some engineers still overlook this issue, he warns
Recently, I had the chance to catch up with a friend who was an aerospace engineer in the 1960s. He worked on some incredible projects, such as the Apollo programme and the F105 Thunderchief supersonic fighter-bomber.
As he recounted over drinks, if bombs from the F105 were dropped even slightly out of sync – measured in mere hundredths of a second – a vibration was initiated that rapidly grew in magnitude through the body of the plane, until it reached a level forcing the pilot to bailout.
This, for me at least, is the new physics textbook classic, overtaking the Tacoma Narrows Bridge, where miscalculated vibrations tore the suspension bridge apart, causing it to collapse into the ocean in 1940.
I haven’t written much about dynamics in these articles, but this conversation reminded me that I ought to do so. All structures have a resonant or natural frequency.
In fact, they generally have quite a few, and these are a property – just like mass, colour or surface finish. You don’t have to do anything to the object for it to have a natural frequency, and it’s a metric that can be quite easily calculated.
Or at least, it can if you’ve got a finite element analysis (FEA) programme.
Degree-level engineering courses spend a good deal of time taking students through how to calculate the natural frequency of a spring/mass system. And I’ve probably spent too long here talking about how finite element models are essentially elaborate arrays of springs. So, if we add a representation of the mass to our array of springs, the natural frequencies can these days be extracted from the whole thing, if not with ease, then at least with speed.
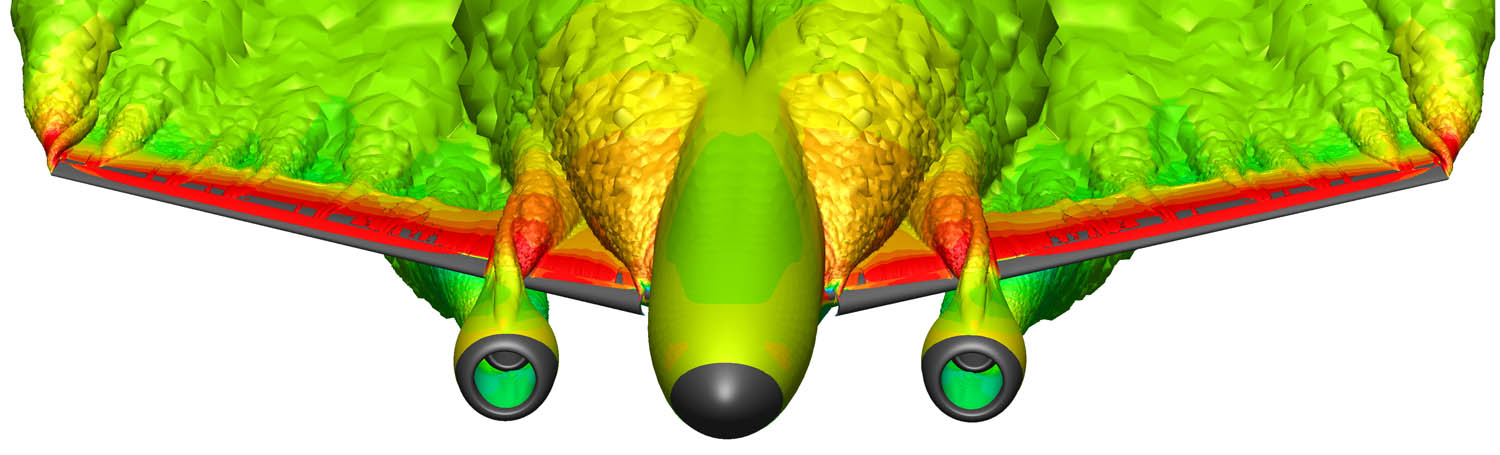
Forewarned is forearmed
For lots of design scenarios, this is critically important information. Knowing at what frequency things will kick off – like an F105 dropping its bomb load asymmetrically or a bridge in Washington State with a curious relationship between its natural frequency and the airflow over its deck – is pretty important. The solution will also give you a mode shape, or a series of mode shapes, which correspond to the natural frequencies.
Modifying a design to shift frequency away from the troublesome range should be a basic part of every design process — but it almost certainly isn’t
Armed with this information, modifying the design to shift frequency away from the troublesome range becomes an intuitive process. It should be a basic part of every design process – but it almost certainly isn’t.
Which brings me neatly to what happens when the world around our design or machine starts getting involved. In the real world, for the dynamics to reveal themselves, something has to be putting energy into the system and, to some extent, there will also be energy escaping the system: excitation and damping.
Generally, it’s easier to assess the first than the second, though both can be added to finite element studies. At this point, the game gets a lot more serious. Earthquakes, industrial machinery and lots of other forcing functions can all be modelled. However, damping remains a problem, because it’s the dissipation of energy, and it takes many forms – friction in joints, heat generated as material is deformed, and so on.
There are many ways energy leaves the system, one being by moving around the fluid surrounding the object. Which takes us back to the Tacoma Narrows Bridge, because not only will fluid damp the vibrations, it can also excite them. If the natural frequency and the flow transients are at the same frequency, the results can be ‘exciting’, to say the least.
If we want to simulate the problem, we enter the world of closely coupled multiphysics. Twenty years ago, there was plenty of talk about multiphysics and FSI. So by now, you might expect it to have become commonplace. But it hasn’t.
So why isn’t fully coupled CFD and structural FEA a regular thing in the world of simulation? In some places, it plainly is, but it’s not exactly run of the mill in everyday simulation workflows, even given the huge increases in compute power now available to everyone, either on their desktop or in the cloud.
Like so many simulation holy grails, usage may well be restricted to those people with a combination of big budgets, big problems and a pretty comprehensive understanding of the physics involved. That would certainly describe the designers of the Century Series jets (including the F105) back in the day. But even here and now, we get regular reminders of what can go wrong in design when vital calculations get neglected – not least with this Formula 1 season’s ‘porpoising’ cars.
Laurence Marks built his first FEA model in the mid-1980s and his first CFD model in the early 1990s. Since then, he’s worked in the simulation industry, in technical, support and management roles. He is currently a visiting research fellow at Oxford Brookes University, involved in a wide range of simulation projects, some of which are focused on his two main areas of interest: life sciences and motorsports